Heat Transfer
Heat transfer is an engineering discipline that concerns the generation, use, conversion, and exchange of heat (thermal energy) between physical systems. In power engineering it determines key parameters and materials of heat exchangers. Heat transfer is usually classified into various mechanisms, such as:
- Heat Conduction. Heat conduction, also called diffusion, occurs within a body or between two bodies in contact. It is the direct microscopic exchange of kinetic energy of particles through the boundary between two systems. When an object is at a different temperature from another body or its surroundings
- Heat Convection. Heat convection depends on motion of mass from one region of space to another. Heat convection occurs when bulk flow of a fluid (gas or liquid) carries heat along with the flow of matter in the fluid.
- Thermal Radiation. Radiation is heat transfer by electromagnetic radiation, such as sunshine, with no need for matter to be present in the space between bodies.
Heat Transfer in Nuclear Engineering – Application
Heat transfer is commonly encountered in engineering systems and other aspects of life, and one does not need to go very far to see some application areas of heat transfer.
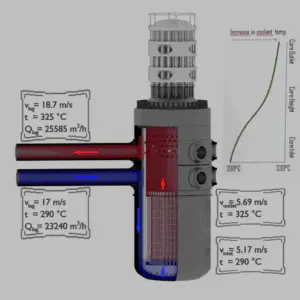
Detailed knowledge of heat transfer mechanisms is also essential for reactor engineers as well as all other engineers. A nuclear power plant (nuclear power station) looks like a standard thermal power station with one exception. The heat source in the nuclear power plant is a nuclear reactor. As is typical in all conventional thermal power stations the heat is used to generate steam which drives a steam turbine connected to a generator which produces electricity. But in nuclear power plants reactors produce enormous amount of heat (energy) in a small volume. The density of the energy generation is very large and this puts demands on its heat transfer system (reactor coolant system). Therefore we have to start by the reactor heat generation and removal from the reactor.
For a reactor to operate in a steady state, all of the heat released in the system must be removed as fast as it is produced. This is accomplished by passing a liquid or gaseous coolant through the core and through other regions where heat is generated. The heat transfer must be equal to or greater than the heat generation rate or overheating and possible damage to the fuel may occur. The nature and operation of this coolant system is one of the most important considerations in the design of a nuclear reactor.
It should be noted that from a strictly nuclear standpoint, there is theoretically no upper limit to the reactor thermal power, which can be attained by any critical reactor having sufficient excess of reactivity to overcome its negative temperature feedbacks. In each nuclear reactor, there is a direct proportionality between the neutron flux and the reactor thermal power. The term thermal power is usually used, because it means the rate at which heat is produced in the reactor core as the result of fissions in the fuel. Moreover, for a short period, a critical reactor does not need to have high excess of reactivity as in case of rapid reactivity excursions.
In short, almost any reactor is able to exceed the ability of heat removal of its coolant system. Beyond this point, the fuel would heat up and can reach very high temperatures. This situation must be avoided by reactor operator and by reactor safety systems. It is essential, that the heat generation – heat removal rate balance must be maintained to prevent these temperatures that might result in the failure of fuel or other structural materials. In reactor engineering, the thermal-hydraulics of nuclear reactors describe the effort involving the coupling of heat transfer and fluid dynamics to accomplish the desired heat removal rate from the core under both normal operation and accident conditions.
We hope, this article, Heat Transfer, helps you. If so, give us a like in the sidebar. Main purpose of this website is to help the public to learn some interesting and important information about thermal engineering.